The Quantum World: How Quantum Chemistry is Changing Our Understanding of Matter
- Hina Agrawal
- Sep 29, 2024
- 6 min read
Chemistry is often regarded as the "central science" because it connects the physical sciences with the life sciences. Traditionally, chemistry has been taught with a focus on atomic theory, chemical reactions, bonding, and the periodic table. However, at the most fundamental level, the behavior of atoms and molecules isn't governed by the rules we experience in our macroscopic world—it is driven by the strange and fascinating principles of quantum mechanics. This quantum realm, which operates on scales smaller than atoms, has been revolutionizing our understanding of chemistry for over a century. But only recently has the field of **quantum chemistry** begun to take center stage, offering deeper insights into the very fabric of matter.
What is Quantum Chemistry?
Quantum chemistry is a branch of chemistry focused on understanding the behavior of electrons, atoms, and molecules using the principles of quantum mechanics. It seeks to explain how particles behave at atomic and subatomic levels by using mathematical equations and models derived from quantum theory. This field deals with the wave-like nature of particles and the probability distributions that describe where an electron is likely to be found around a nucleus. Unlike classical physics, where objects have defined positions and velocities, quantum systems are inherently uncertain, leading to mind-bending phenomena such as superposition and entanglement.
In essence, quantum chemistry is about applying quantum mechanics to chemical systems to explain how and why chemical reactions occur, how bonds form, and how energy is exchanged between matter.
The Birth of Quantum Chemistry: Schrödinger and Heisenberg
To fully understand the significance of quantum chemistry, we need to revisit the early 20th century when quantum theory first emerged. Two prominent physicists, Erwin Schrödinger and Werner Heisenberg, developed two different yet complementary models to describe quantum behavior.
- Schrödinger's wave mechanics: Schrödinger formulated a mathematical equation, now famously known as the Schrödinger equation, that describes how the quantum state of a system changes over time. In this approach, particles like electrons are considered wave functions, and their behavior can be described using probability distributions. This equation is fundamental in predicting the behavior of electrons in atoms and molecules.
- Heisenberg's uncertainty principle: Werner Heisenberg introduced the uncertainty principle, which states that it is impossible to know both the exact position and momentum of a particle simultaneously. The more precisely we know one, the less precisely we can know the other. This principle is central to quantum mechanics and challenges our classical understanding of particles moving in well-defined paths.
Key Principles in Quantum Chemistry
To grasp the power of quantum chemistry, we need to understand a few key principles that differ from classical physics:
1. Wave-Particle Duality: At the quantum level, particles such as electrons exhibit both particle-like and wave-like behavior. This dual nature allows quantum particles to exist in multiple states simultaneously, which is key to understanding how electrons behave in atoms.
2. Superposition: In quantum systems, particles can exist in a superposition of states, meaning they can be in multiple places or have multiple energies at the same time. This leads to unique behaviors in chemical bonding and molecular structure.
3. Quantum Tunneling: In certain cases, particles can "tunnel" through energy barriers that would be insurmountable according to classical physics. This phenomenon is crucial in many chemical reactions, particularly in biological systems such as enzymes.
4. Quantum Entanglement: When two particles become entangled, the state of one particle is instantly connected to the state of the other, no matter how far apart they are. Although this phenomenon is more famous in the field of quantum computing, it has implications for understanding complex molecular interactions.
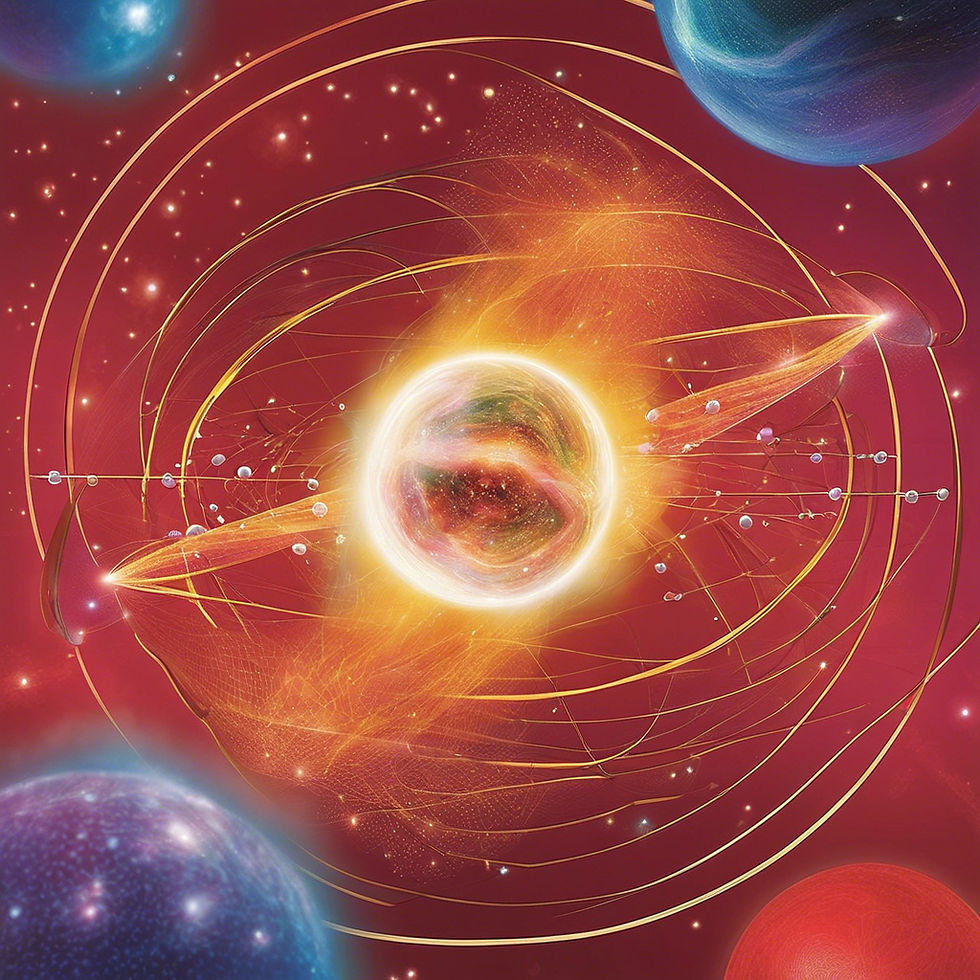
Quantum Chemistry and Chemical Bonding
One of the most profound contributions of quantum chemistry has been in the understanding of chemical bonding. Classical chemistry explains chemical bonds using models such as the ionic, covalent, and metallic bonds. However, quantum chemistry goes deeper by explaining the behavior of electrons during bond formation through quantum mechanical models like molecular orbitals and hybridization.
- Molecular Orbital Theory: In quantum chemistry, molecular orbital theory offers a more nuanced explanation of how atoms bond. Rather than thinking of electrons in terms of individual atoms, the theory posits that electrons are distributed in molecular orbitals that span across the entire molecule. These orbitals can either be bonding (lower energy, stabilizing) or anti-bonding (higher energy, destabilizing). The distribution of electrons in these orbitals determines the stability and reactivity of molecules.
- Valence Bond Theory and Hybridization: Another approach from quantum chemistry is valence bond theory, which states that bonds form when atomic orbitals overlap. The concept of hybridization explains how atomic orbitals combine to form new orbitals, such as sp, sp2, and sp3, each with different geometrical orientations, which play a role in the shapes and bonding patterns of molecules.
Quantum Chemistry and Spectroscopy
One of the most important applications of quantum chemistry is in the field of spectroscopy, where light is used to study the interaction of energy with matter. Quantum theory allows chemists to understand how different molecules absorb, emit, or scatter light at various frequencies. By applying the Schrödinger equation to molecules, quantum chemistry can predict the specific energy levels that an electron can occupy. This, in turn, allows scientists to deduce the structure and behavior of molecules from spectroscopic data.
- Infrared Spectroscopy (IR): Quantum chemistry helps explain how molecules vibrate and rotate when they absorb infrared light. Each molecule has unique vibrational modes that correspond to different energy levels, allowing chemists to identify molecular structures based on their IR spectra.
- Nuclear Magnetic Resonance (NMR): NMR spectroscopy is widely used in organic chemistry to determine the structure of complex molecules. Quantum chemistry helps explain how atomic nuclei, such as hydrogen, respond to magnetic fields. By studying the quantum behavior of these nuclei, chemists can gain insights into the environment and connectivity of atoms within a molecule.
Quantum Chemistry in Action: Drug Design and Material Science
One of the most exciting frontiers of quantum chemistry is its application in drug design and material science. In recent years, computational quantum chemistry has allowed scientists to simulate complex molecular systems and predict how they will interact with one another, without the need for costly and time-consuming experiments.
- Drug Design: The pharmaceutical industry is increasingly using quantum chemistry to simulate how potential drug molecules interact with biological targets, such as proteins or DNA. This allows researchers to design more effective and targeted drugs. For example, quantum chemical methods have been used to model the binding of small molecules to enzymes, helping researchers to develop inhibitors for diseases like cancer and Alzheimer's.
- Material Science: Quantum chemistry is also critical in the development of new materials with specific properties, such as superconductors, semiconductors, and polymers. By understanding how electrons behave in these materials, scientists can design substances that exhibit specific electronic, optical, or mechanical properties. Quantum simulations have been essential in the development of materials used in solar cells, batteries, and even quantum computers.
The Future: Quantum Computers and Chemistry
Quantum chemistry has always relied on computational methods, but the advent of **quantum computers** promises to revolutionize this field even further. Classical computers are limited by their inability to simulate the vast number of possible quantum states that particles can occupy. Quantum computers, on the other hand, can process multiple states simultaneously thanks to the principles of superposition and entanglement. This will allow chemists to simulate complex molecular systems with unparalleled accuracy.
For example, simulating the behavior of large biological molecules, such as proteins, requires enormous computational power because of the number of possible interactions between electrons and atoms. With quantum computers, scientists will be able to model these interactions much more precisely, leading to breakthroughs in fields like personalized medicine, nanotechnology, and energy storage.
Conclusion
Quantum chemistry is transforming our understanding of matter at the most fundamental level. By merging the abstract principles of quantum mechanics with the practical needs of chemistry, this field has allowed scientists to gain unprecedented insights into the behavior of atoms and molecules. From explaining the intricacies of chemical bonding to revolutionizing fields such as drug design and material science, quantum chemistry is at the forefront of scientific progress. As quantum computers become more advanced, the potential of this field will only continue to grow, unlocking new possibilities for how we understand and manipulate the molecular world.
In this quantum-driven future, chemistry, far from being a static science of elements and reactions, will evolve into a dynamic and limitless exploration of the smallest—and most powerful—building blocks of the universe.
Comments